Radiation Detectors Explained: Principles, Technologies, and Applications
Explore how different radiation detectors work, from gas-filled tubes and scintillators to semiconductors and passive dosimeters. Learn the physics and applications in this accessible guide for an educated audience.
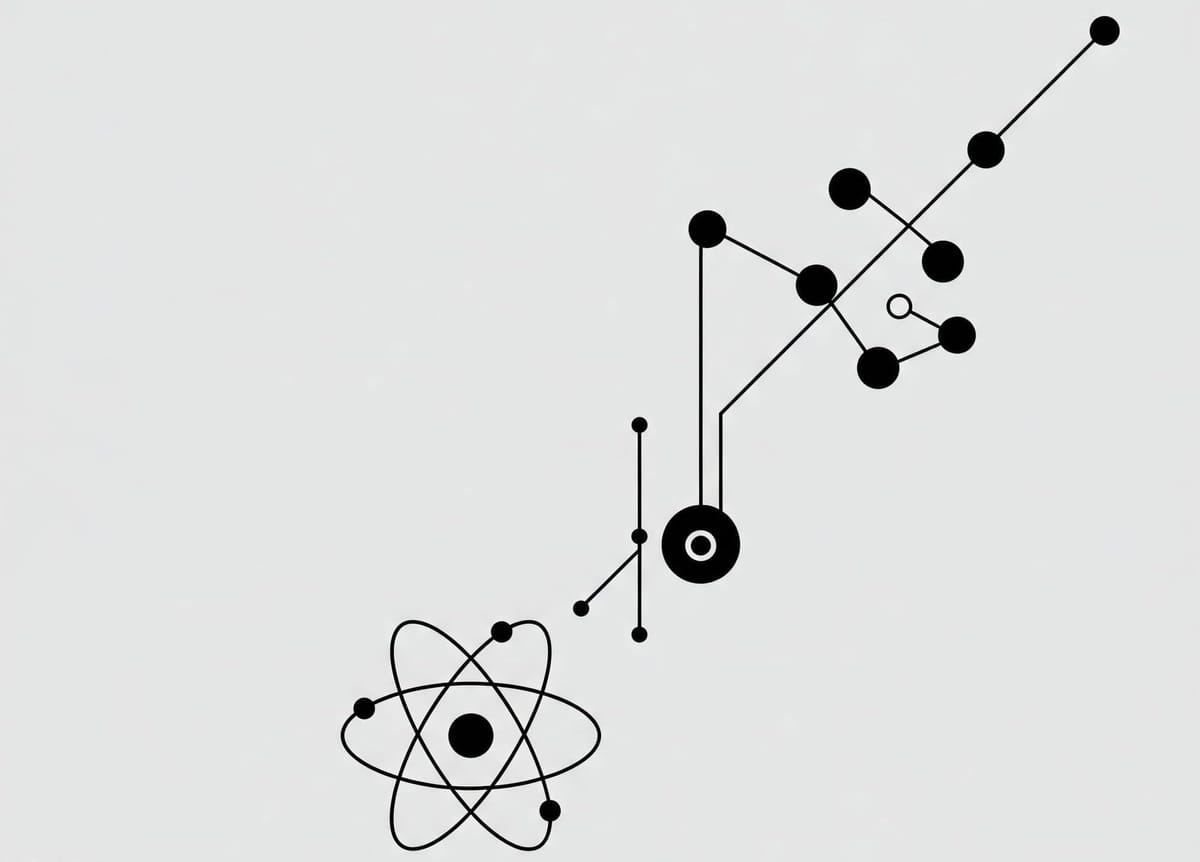
Ever thought about the invisible forces shaping our world? From the diagnostic tools in hospitals to the safety checks at nuclear power plants, our ability to detect ionizing radiation is fundamental. This isn't science fiction; it's a sophisticated science that allows us to perceive energy forms – like alpha particles, beta particles, gamma rays, and neutrons – that are otherwise hidden from our senses. But how exactly do we make the invisible, visible?
It all boils down to a fundamental interaction: When sufficiently energetic radiation strikes matter, it can knock electrons out of atoms (ionization) or boost them to higher energy states (excitation). Imagine an energetic cue ball (radiation) striking a rack of billiard balls (atoms), scattering electrons like pins. Various radiation detection technologies cleverly harness these interactions to create measurable signals. Let's dive into how these detectors work.
The Detector Families: A Toolkit for the Invisible
Different detectors are like different tools in a high-tech toolbox, each suited for specific tasks. They primarily fall into a few main categories:
1. Gas-Filled Detectors: Making Gas Tell the Story
These detectors are the classic workhorses, essentially gas-filled chambers with electrodes. When radiation passes through, it ionizes the gas. The magic lies in the voltage applied across the electrodes, which dictates how the detector behaves:
- Ionization Chambers (The Steady Stream): At low voltages, the detector simply collects all the primary ion pairs created by radiation. It measures a continuous, tiny electrical current proportional to the radiation intensity or dose rate. Analogy: Imagine gently collecting raindrops in a bucket—the rate the bucket fills tells you how hard it's raining. Ionization chambers are reliable for measuring dose rates but not super sensitive to faint signals.
- Proportional Counters (Measuring the Ripple): Things get more interesting at medium voltages. The initial electrons gain enough energy to cause more ionization – a controlled "mini-avalanche". The resulting electrical pulse is proportional to the energy the original radiation deposited. Analogy: Now, each raindrop creates a ripple in the bucket, and the size of the ripple tells you something about the raindrop's size (energy). This allows for spectroscopy – measuring the radiation's energy.
- Geiger-Müller (GM) Counters (The Alarm Bell): Crank up the voltage further, and any single ionizing event triggers a full-blown electrical avalanche across the entire gas volume. It's like one raindrop setting off a flood alarm. This produces a large, unmistakable pulse, making GM counters incredibly sensitive for detecting the presence of radiation (often giving the famous "click"). However, all pulses are the same size, so it can't tell you the radiation's energy, and it gets overwhelmed (due to dead time) at high radiation rates.
2. Scintillation Detectors: When Radiation Makes Light Work
Imagine materials that sparkle when hit by radiation! That's the essence of scintillators.
- Principle: Certain materials (crystals like NaI(Tl), plastics, liquids) absorb radiation energy and instantly re-emit it as tiny flashes of light (scintillation). Often, the brighter the flash, the more energy the radiation deposited.
- How it Works: These faint flashes are captured by highly sensitive light detectors, like Photomultiplier Tubes (PMTs) or Silicon Photomultipliers (SiPMs). PMTs are like incredible amplifiers for light, using electron multiplication stages to turn a few photons into a measurable electrical pulse. SiPMs are their modern, compact, solid-state cousins.
- Use: Because they can be very sensitive and often relate light output to energy, they are stars in gamma spectroscopy (identifying isotopes), medical imaging (PET/SPECT scans), and sensitive surveys. Analogy: Think of radiation striking the scintillator like hitting a tiny drum that flashes light – the brightness tells you how hard it was hit, and the PMT/SiPM is the microphone amplifying that faint flash.
3. Semiconductor Detectors: Solid-State Precision
These detectors bring the precision of semiconductor technology to radiation detection.
- Principle: Instead of gas or scintillators, they use solid semiconductor materials (like Silicon or Germanium). Radiation striking the semiconductor creates electron-hole pairs (mobile negative and positive charges). An applied electric field sweeps these charges away, creating an electrical pulse.
- Why So Precise? Creating an electron-hole pair in a semiconductor takes significantly less energy than an ion pair in gas. This means more charge carriers are generated for the same amount of deposited energy, allowing for much finer distinctions in energy measurement – hence, excellent energy resolution.
- Key Players: High-Purity Germanium (HPGe) detectors offer unparalleled energy resolution for gamma rays but need cooling. Silicon (Si) detectors are great for charged particles. Materials like Cadmium Telluride (CdTe) and CZT perform well at room temperature and are ideal for portable devices. Diamond offers incredible radiation hardness.
- Analogy: Imagine sorting grains of sand instead of pebbles – the finer resolution of semiconductors allows for much more precise energy "sorting."
4. Passive Dosimeters: The Silent Recorders
Not all detection needs to be real-time. Passive dosimeters quietly measure the total radiation dose accumulated over time. Think of them as radiation exposure journals.
- TLDs and OSLDs (The Glow Sticks): Thermoluminescent (TLD) and Optically Stimulated Luminescence (OSLD) dosimeters use special crystals that trap electrons when exposed to radiation. Later, stimulating the crystal with heat (TLD) or light (OSLD) releases the trapped energy as a measurable glow, proportional to the total dose received. Analogy: It is Like a glow-in-the-dark sticker absorbing energy and releasing it later when prompted. They are staples in personal radiation monitoring badges.
- Film Badges (The Old School Photo): These use photographic film that darkens when exposed to radiation. Filters help estimate radiation type/energy. They provide a permanent record but are less accurate than modern TLDs/OSLDs.
- Track-Etch Detectors (The Damage Trail): Certain plastics record the passage of heavy particles (like alpha particles or neutrons) as microscopic damage tracks. Chemical etching makes these tracks visible for counting. This is great for specific particle detection, like radon monitoring.
Beyond the Mainstream: Specialized Tools
The world of radiation detection also includes specialized instruments:
- Neutron Detectors: Since neutrons are neutral, they're detected indirectly through nuclear reactions they induce in specific materials (like Helium-3, Boron-10, Lithium-6) that release detectable charged particles.
- Cherenkov Detectors: For particles moving faster than light in a medium! These particles create a shockwave of light (Cherenkov radiation), like an optical sonic boom. Used in high-energy physics and neutrino detection.
- Bolometers: Ultra-sensitive thermometers that measure the tiny heat increase when radiation is absorbed. Often used in astronomy and requires extreme cooling.
- Microchannel Plates (MCPs): Arrays of millions of tiny amplifying channels, used to detect single particles or photons with high precision in position and time.
Why This Technology Matters
Radiation detection is indispensable for safeguarding nuclear workers and ensuring the accuracy of cancer radiation therapy to enable discoveries in astrophysics and help us understand fundamental physics. It allows us to monitor our environment, protect national security, and push the boundaries of science and medicine. The continuous drive for better sensitivity, resolution, and speed in these detectors fuels innovation across countless fields.
The Takeaway: An Ever-Evolving Window into the Invisible
The diverse family of radiation detectors provides humanity with essential tools to interact with and understand the invisible energetic world around us. Each technology, born from fundamental physics principles, offers a unique way to measure radiation, tailored to specific needs. As technology advances, we can expect even more sophisticated detectors, enhancing our safety, deepening our scientific knowledge, and improving our lives in ways yet to be imagined.
Which area (like medicine, environmental safety, or fundamental research) will gain the most from the next generation of radiation detectors?